Visual Abstract
Abstract
Breast cancer, particularly aggressive subtypes like triple-negative breast cancer (TNBC), presents significant treatment challenges, with conventional methods often causing severe side effects. This review highlights the potential of gold nanoparticles (AuNPs) in innovative breast cancer treatments, particularly photodynamic therapy (PDT) and photothermal therapy (PTT). AuNPs are promising due to their ability to efficiently absorb near-infrared (NIR) light, converting it into localized heat for PTT. This approach selectively destroys cancer cells by exploiting the enhanced permeability and retention (EPR) effect, which allows AuNPs to accumulate in tumor tissues. Additionally, AuNPs can deliver photosensitizers in PDT, generating reactive oxygen species (ROS) upon light exposure, further enhancing cancer cell destruction. The synergistic combination of PDT and PTT offers improved targeting and therapeutic efficacy with reduced side effects. AuNPs can also augment chemotherapy by enhancing drug delivery and addressing resistance. However, challenges remain, including biocompatibility, production scalability, and clinical translation. This review provides a comprehensive overview of the advantages, limitations, and prospects of utilizing AuNPs in breast cancer phototherapy. Emphasis is placed on further research to optimize nanoparticle design, improve targeting efficiency, and fully address safety concerns to realize their clinical potential.
Introduction
Breast cancer is the second most common cancer among women globally, following non-melanoma skin cancers, and remains the leading cause of cancer-related deaths among women. In 2020, 2.3 million new cases were diagnosed, resulting in 684,996 deaths, representing 15.5% of female cancer mortalities. Despite advancements in treatment and a generally favorable prognosis, managing breast cancer is challenging due to the diverse nature of malignant cells in mammary epithelial tissue. Aggressive subtypes such as inflammatory breast cancer (IBC) and triple-negative breast cancer (TNBC) pose additional challenges. Invasive forms, like invasive ductal carcinoma (IDC) and invasive lobular carcinoma (ILC), are more aggressive but less common compared to non-invasive types like ductal carcinoma in situ (DCIS). This diversity emphasizes the need for innovative research and approaches to address the disease’s complexity [1,2].
Current treatments include surgery, radiation, chemotherapy, immunotherapy, targeted therapy, hormone therapy, stem cell transplants, and precision medicine. However, these methods often cause side effects, such as anemia, appetite loss, bleeding, bruising, constipation, delirium, diarrhea, swelling, and fatigue [3].
Hyperthermia has emerged as a promising adjunct in breast cancer treatment, particularly for aggressive subtypes like TNBC and IBC, where conventional therapies often fall short. This technique leverages controlled heat to selectively target malignant cells, enhancing drug delivery, sensitizing tumors to radiotherapy, and activating immune responses. Historically rooted in ancient medicine and systematically explored since the 1970s, hyperthermia has demonstrated efficacy in improving outcomes for various cancers, especially recurrent or treatment-resistant tumors. Integrating hyperthermia with personalized medicine and combination therapies offers a valuable strategy to overcome therapeutic resistance and improve patient outcomes while minimizing additional side effects [4–6]. The use of gold nanoparticles (AuNPs) in breast cancer treatment has gained significant attention due to their unique physicochemical properties, including high surface area-to-volume ratio, biocompatibility, and tunable optical and thermal characteristics. These features make AuNPs particularly effective for targeted drug delivery, phototherapy, and imaging applications. Despite the advancement of conventional therapies, critical gaps remain in addressing treatment resistance, minimizing systemic toxicity, and improving tumor specificity. Gold nanoparticles offer a promising avenue to bridge these gaps by enabling precision therapy that can be tailored to the heterogeneous nature of breast cancer. Additionally, AuNPs’ ability to enhance the efficacy of hyperthermia and synergize with other therapeutic modalities underscores their potential to improve outcomes for patients with aggressive or treatment-resistant breast cancer subtypes [7]. However, significant scientific gaps remain, particularly regarding the long-term safety profiles of AuNPs, their potential toxicity in major organs, and the optimization of their synthesis and functionalization for clinical applications. By addressing these gaps, ongoing research into AuNPs could significantly advance the field of breast cancer treatment, offering new avenues for effective and personalized therapies.
Core Principles and Mechanisms of Hyperthermia in Cancer Therapy
Hyperthermia, an adjunctive cancer therapy, utilizes elevated temperatures (40–43°C) to target tumor tissues selectively, exploiting cancer cells’ heightened sensitivity to heat compared to normal tissues. Established over four decades as an effective radiosensitizer and chemosensitizer, hyperthermia enhances the efficacy of radiotherapy and chemotherapy by inducing direct DNA damage (single- and double-strand breaks) and impairing DNA repair pathways. It also disrupts cellular proteins, leading to denaturation and aggregation, particularly in nuclear proteins critical for DNA replication and repair [8, 9]. While heat shock proteins (HSPs) upregulate in response, potentially causing thermotolerance, their management is crucial to sustaining hyperthermia’s effectiveness. Hyperthermia induces cell death via apoptosis (through intrinsic mitochondrial and extrinsic receptor-mediated pathways) and necrosis under prolonged or higher heat exposure, leading to tumor disruption. These multifaceted effects make hyperthermia a potent tool for treating various cancers, especially those resistant to standard therapies [10–12].
Advancements in Hyperthermia Techniques: From Traditional Methods to Nanotechnology-Based Innovations
Hyperthermia therapy has advanced significantly with the development of traditional and nanotechnology-based methods. Traditional techniques include microwave heating, radiofrequency ablation (RFA), and high-intensity focused ultrasound (HIFU) [13]. Microwave heating is a non-invasive cancer treatment that uses microwave energy to generate localized heat, damaging cancer cells or enhancing other therapies. It is effective for hyperthermia (40–45°C) or thermal ablation (>50°C) and is particularly useful for small or hard-to-reach tumors. Delivered minimally invasively, it offers precise targeting, reduced healthy tissue damage, and compatibility with other treatments [14]. RFA is a minimally invasive technique that uses radio waves through needle electrodes to heat and destroy tumors, commonly used for liver and kidney cancers. It is guided by imaging techniques to ensure precision. HIFU uses focused ultrasound waves to target and destroy cancer cells non-invasively, especially effective for localized prostate cancer. Both methods offer precise, targeted treatment with minimal side effects and are less invasive alternatives to traditional surgery [15]. Nanotechnology has further enhanced hyperthermia by introducing nanomaterials as heating agents, allowing for more controlled and localized heat generation using external stimuli like magnetic fields, and infrared radiation (Phototherapy). Advances in nanotechnology enable precise temperature monitoring, improving the precision of thermal treatments. The integration of nanotechnology, particularly through magnetic-thermal and photo-induced thermal therapies, has revitalized hyperthermia by enhancing its ability to induce therapeutic effects, such as altering tissue elasticity and blood flow. Ongoing research aims to optimize localized heating and understand heat-induced cellular changes, solidifying hyperthermia as a promising, minimally invasive cancer treatment [16].
Phototherapy: Introduction and Mechanisms
Phototherapy is an advanced approach to cancer treatment that uses light of specific wavelengths to induce therapeutic effects. The two main modalities of phototherapy are photodynamic therapy (PDT) and photothermal therapy (PTT), each operating through distinct mechanisms [17]. The PDT method involves using light, a photosensitizer (PS), and oxygen to produce cytotoxic reactive oxygen species (ROS) that damage cellular components. Upon light activation, the PS undergoes energy transfer reactions to generate singlet oxygen or other ROS, triggering apoptosis, necrosis, or inflammatory responses. PDT’s precision minimizes damage to healthy tissues, making it ideal for treating tumors near critical structures, such as the head, neck, and bladder. Advances in photosensitizer design, such as oxygen-independent PS, are expanding its therapeutic applications [18]. PTT employs near-infrared (NIR) light and photothermal agents to convert light energy into heat, raising the temperature within the tumor microenvironment. This localized hyperthermia leads to cancer cell death through apoptosis or necrosis while sparing surrounding healthy tissues. The development of advanced nanomaterials has enhanced PTT’s efficiency and specificity, reducing side effects and improving therapeutic outcomes [18,19]. PDT and PTT offer precise spatiotemporal control, lower off-target toxicity, and the ability to overcome chemotherapy resistance by interfering with tumor signaling pathways. Additionally, they use non-ionizing radiation, reducing the risk of secondary cancer compared to conventional radiotherapy.
Role of Gold Nanoparticles in Phototherapy
AuNPs have emerged as powerful tools in PDT and PTT due to their unique optical and physicochemical properties. Their ability to interact with light through surface plasmon resonance (SPR) enables efficient energy conversion and enhanced therapeutic effects [16]. In PTT AuNPs absorb NIR light (650–850 nm) and convert it into localized heat via SPR, leading to rapid temperature elevation in tumor tissues. This property allows precise targeting of cancer cells while minimizing harm to adjacent healthy tissues. By optimizing their size, shape, and surface characteristics, AuNPs achieve higher photothermal conversion efficiency. The enhanced permeability and retention (EPR) effect further aids their accumulation in tumor sites, improving treatment outcomes [20, 21]. The shape of gold nanoparticles significantly influences their optical properties and, consequently, their effectiveness in PTT. For instance, gold nanorods (AuNRs) have been shown to possess superior photothermal conversion efficiencies compared to spherical AuNPs due to their elongated shape that allows for stronger SPR absorption in the NIR region. Research indicates that AuNRs can achieve conversion efficiencies ranging from 39% to 56%, depending on their aspect ratio and the surrounding medium. Additionally, gold nanoflowers (AuNFs) exhibit a high photothermal conversion efficiency of about 74%, attributed to their complex structure that enhances light absorption [22]. The size of AuNPs also plays a crucial role in determining their therapeutic efficacy. Smaller nanoparticles tend to have higher surface area-to-volume ratios, which can enhance their interaction with light and biological tissues. However, larger nanoparticles may provide better stability and prolonged circulation times in the bloodstream. Studies have demonstrated that the optimal size for achieving maximum photothermal effect typically falls within the range of 50 to 100 nm. This size range is associated with improved tissue penetration and reduced scattering losses, ultimately leading to more effective heating of tumor cells [23].
In PDT AuNPs can act as photosensitizers or enhance existing PS performance. Upon light activation, in the NIR range (700–800 nm), they facilitate the generation of ROS, including singlet oxygen (1O2), superoxide anions (O2−), and hydroxyl radicals (OH⋅) [24, 25]. These ROS induce oxidative stress and cellular damage, effectively targeting tumor cells while sparing normal tissues. The biocompatibility, tunable optical properties, and functionalization potential of AuNPs make them suitable for advanced PDT applications. The efficiency of this process is enhanced by the EPR effect, allowing AuNPs to preferentially accumulate in tumor sites due to their leaky vasculature. Studies have demonstrated that specific configurations of AuNPs, such as gold nano bipyramids, exhibit superior photothermal conversion efficiency, enabling effective tumor ablation with lower laser power and shorter exposure times compared to traditional methods [26, 27]. The integration of AuNPs in phototherapy has shown significant promise in breast cancer treatment, particularly for challenging cases like TNBC. This section provides a review of recent studies leveraging gold nanomaterials in the photothermal therapy of breast cancer, highlighting their innovative applications, therapeutic efficacy, and potential to revolutionize treatment paradigms (Figure 1).
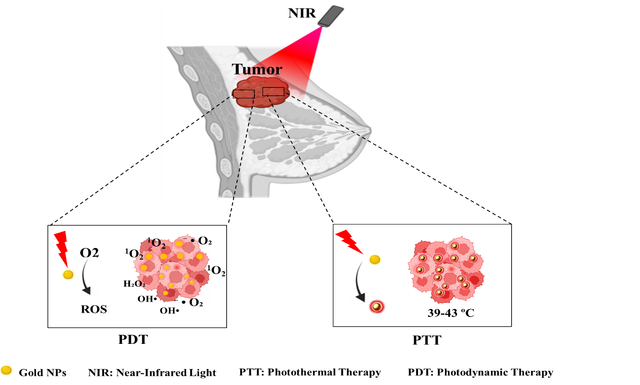
Gold Nanoparticle-Based Photothermal for Breast Cancer Treatment
Numerous studies have explored the potential of AuNPs in combination with different stimuli to enhance therapeutic outcomes in breast cancer treatment. In one study, AuNPs combined with green laser light were used to enhance the efficacy of doxorubicin (DOX) in breast cancer treatment, achieving synergistic effects that significantly reduced cell survival. The combination of AuNPs and DOX under light irradiation resulted in improved therapeutic outcomes by enhancing drug delivery and activation at the tumor site. This approach demonstrates the potential for combining chemotherapy with PTT, improving both drug efficacy and cancer cell targeting. The study highlights the significance of light-mediated nanoparticle activation for achieving greater therapeutic efficiency in cancer treatment [28]. Similarly, Cheng et al. introduced a targeted microwave-activated gold nanosystem to advance the development of photothermal conversion agents for treating TNBC. This system features a gold nanocage (AuNC) as an effective photothermal conversion agent combined with an HSP monoclonal antibody to precisely target HSPs. Initially, microwave irradiation of 4T1 cells was shown to elevate HSP70 expression, a finding validated through western blot analysis. Subsequently, the designed cmHSP-AuNC nanosystem was employed in PTT to enhance the accumulation of photothermal agents within TNBC cells, thereby improving PTT efficacy. Overall, based on both in vivo and in vitro findings, this research could pave the way for further advancements in tumor-targeted PTT strategies [29]. Building upon these findings, the present study developed a novel CSC-specific targeted nano platform for the effective eradication of CSCs and prevention of tumor relapse. This system integrates retinoic acid (RA)-loaded gold nanostars with dendritic polyglycerol (GNSs-dPG), further functionalized with hyaluronic acid (HA) to enable multivalent and CSC-specific targeting. The nano platform exploits RA-induced differentiation to suppress CSC self-renewal capacity, while photothermal therapy (PTT) ensures efficient tumor ablation. This dual-mode approach demonstrated a synergistic effect, significantly inhibiting tumor growth, reducing stemness gene expression, and diminishing CSC-driven tumorsphere formation. In vivo results confirmed the effective elimination of CSCs and tumor growth inhibition, showcasing the nanoplatform’s superior potential for targeted cancer therapy and relapse prevention (Figure 2) [30].
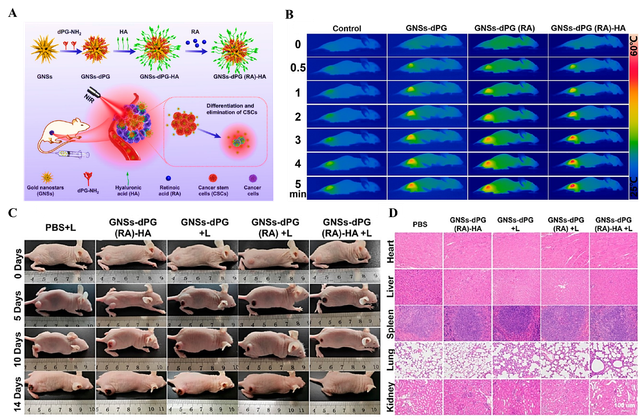
Additionally, Zeng et al. proposed an innovative approach by synthesizing Agcore@Aushell nanostars coupled with a Raman reporter molecule, DTTC, to enhance in vivo surface-enhanced Raman spectroscopy (SERS) imaging and NIR-induced PTT for breast cancer treatment. The dual-phase DTTC configuration significantly amplified the SERS signal, while the gold-coated nanostar structure minimized cytotoxicity. The synthesized Ag@Au-DTTC nanostars demonstrated high photothermal conversion efficiency and exceptional ability to enhance SERS signals in cells and tumors. When administered to mice with MCF–7 tumors and subjected to 808 nm laser irradiation, the nanostars achieved a 5% reduction in cell survival and complete tumor volume disappearance after 14 days of treatment. These findings underscore the potential of Ag@Au-DTTC nanostars as a multifunctional platform, combining NIR-induced PTT and advanced imaging techniques for efficient breast cancer therapy (Figure 3) [31]. In another study by Higbee-Dempsey et al., a nanocluster comprising gold nanoparticles and indocyanine green (ICG) was developed for both PTT and photoacoustic (PA) imaging of breast cancer. The researchers demonstrated that the ICG-AuNP nanocluster, when injected into an orthotopic animal model of triple-negative breast cancer, effectively generated heat under NIR irradiation and allowed for tumor visualization via PA imaging. This approach not only successfully eliminated tumors but also led to prolonged animal survival, proving the dual utility of the nanocluster in both diagnostic imaging and treatment. The study underscores the potential of combining diagnostic and therapeutic modalities in a single nanoparticle system for more comprehensive cancer management [32]. Additionally, a recent study published in Nature Scientific Reports explored the enhancement of chemotherapy using AuNPs. By inducing localized hyperthermia through AuNPs under light irradiation, the researchers observed a significant increase in the efficacy of DOX against breast cancer cells. The combination therapy showed a marked reduction in tumor viability compared to treatments using either modality alone, highlighting the synergistic effect of combining chemotherapy and PTT. This study emphasizes the importance of integrating PTT with conventional chemotherapy to overcome the limitations of standard cancer treatments and improve therapeutic outcomes [21]. A related study developed a novel nanoparticle-based drug delivery system using zinc phthalocyanine (ZnPc) and AuNPs encapsulated in liposomes. This system utilized the hyperthermic effects of AuNPs under LED light to trigger the on-demand release of ZnPc, enabling precise drug delivery to cancer cells. The combination of localized hyperthermia and the photodynamic effect of ZnPc created a synergistic therapeutic outcome, inhibiting up to 86.7% of breast cancer cells (MCF–7 cell line) under red light irradiation. In vivo experiments demonstrated high tumor accumulation and significant antitumor effects, suggesting that this novel system could provide a highly efficient platform for combined photothermal and photodynamic therapy. This study illustrates the potential of integrating multiple therapeutic modalities in a single nanoparticle system for more effective and targeted cancer treatment [33]. Another promising approach to overcoming the limitations of traditional chemotherapy is the development of multifunctional co-delivery systems that combine chemotherapeutic agents with PTT to enhance anticancer efficacy. One such study focused on the co-delivery of camptothecin (CPT) and AuNPs, creating a multifunctional nanocarrier system. The system involved the decoration of camptothecin nanocrystals (CPT NCs) with AuNPs and the addition of a hyaluronic acid (HA) layer, which served as a stabilizing, targeting, and hydrophilic agent. This nanocarrier exhibited favorable physicochemical properties, including optimal size, stability, and photothermal efficiency. The system demonstrated enhanced biocompatibility, selectivity, and intracellular uptake, while significantly improving cytotoxicity and apoptosis induction. The synergistic effects of AuNPs, CPT, near-infrared irradiation, and pH/photothermal-triggered drug release contributed to its effectiveness. Moreover, the mechanism underlying the cytotoxic effects involved mitochondrial dysfunction, oxidative stress, DNA fragmentation, and the activation of proapoptotic genes such as p53 and Bax, while suppressing the antiapoptotic Bcl–2 gene. This study illustrates the potential of combining chemotherapy and PTT in a single nanoplatform to not only improve cancer treatment but also overcome issues such as drug resistance and cancer stem cells (CSCs), which are often responsible for tumor relapse and metastasis [34].
Challenges and Solutions in Gold Nanoparticle-Based Photothermal Therapy for Breast Cancer
AuNPs have shown significant promise in the treatment of breast cancer, particularly through PTT and PDT. However, several challenges hinder their widespread clinical application, including issues related to biocompatibility, production scalability, and cost-effectiveness. A primary concern with AuNPs is their biocompatibility. Despite their potential for drug delivery and imaging, studies have raised alarms about their toxicity to vital organs like the liver and kidneys. AuNPs have been shown to induce oxidative stress and inflammatory responses in cells, which raises questions about their long-term safety in clinical settings [7, 35]. For instance, a study demonstrated that after administering 20 nm spherical AuNPs to mice, significant accumulation was observed in the liver, spleen, and kidneys over a period of 120 days.
Specifically, there was a 39% reduction in liver nanoparticle levels but a marked increase in both the spleen (53%) and kidneys (150%), indicating potential long-term retention and associated toxicity [36]. To mitigate the risks associated with AuNPs while leveraging their therapeutic potential, several strategies can be employed. For example, altering the surface chemistry of AuNPs can enhance their biocompatibility and reduce toxicity. Coating nanoparticles with biocompatible materials like polyethylene glycol (PEG) may help minimize immune responses and enhance clearance from the body. Developing controlled release mechanisms for drug delivery can help limit the exposure duration of AuNPs in vital organs, potentially reducing their toxic effects while maintaining therapeutic efficacy [37]. Conducting long-term in vivo studies is essential to understand the full scope of AuNPs’ biological effects. This includes assessing different sizes, shapes, and surface modifications of nanoparticles to determine optimal formulations that minimize toxicity while maximizing therapeutic benefits [38, 39]. Combining AuNPs with antioxidants may help mitigate oxidative stress induced by these nanoparticles. This dual approach could enhance therapeutic outcomes while protecting vital organs from damage [40]. Another major challenge is achieving selective targeting of tumor cells. While AuNPs can exploit the enhanced permeability and retention (EPR) effect to accumulate in tumors, their distribution can be uneven within the tumor microenvironment. This heterogeneity can result in suboptimal therapeutic outcomes. Factors like the size, shape, and surface modifications of AuNPs significantly influence their biodistribution and cellular uptake. Therefore, careful design is crucial to enhance targeting efficiency [41]. Optimizing the size, shape, and surface modifications of AuNPs can improve their biodistribution and uptake in tumor cells, addressing the tumor’s heterogeneity [42, 43]. Adding targeting ligands that bind to tumor-specific markers can increase the selectivity of AuNPs for cancer cells, minimizing off-target effects [44]. Combining AuNPs with immunotherapy or other treatments can enhance therapeutic efficacy and exploit the EPR effect more effectively [45].
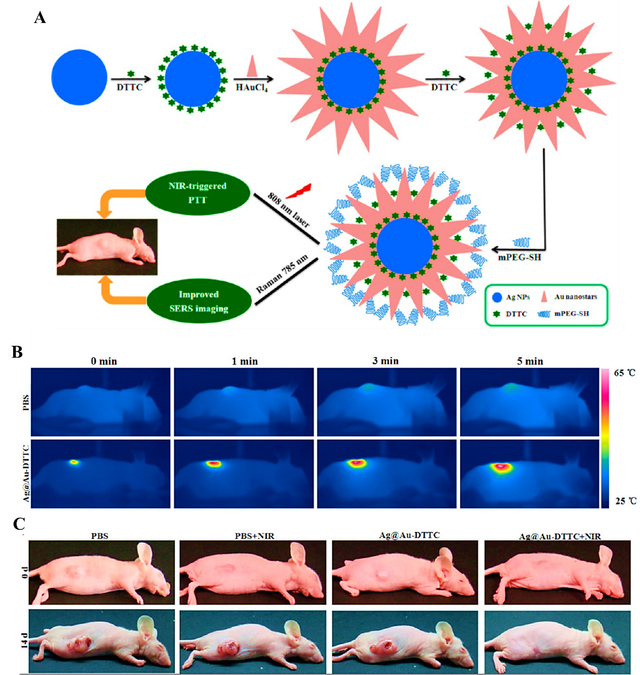
The mechanisms by which AuNPs exert their therapeutic effects are still not fully understood. While AuNPs are known to enhance local heating during PTT by converting light into heat, the exact biological pathways involved in cell death after treatment remain unclear. This lack of understanding complicates the optimization of treatment protocols [25, 46]. Further research into the molecular pathways, especially the role of oxidative stress and cellular signaling, will be crucial for improving the efficacy of PTT and PDT. Additionally, employing advanced imaging techniques to track AuNP behavior in vivo will provide valuable insights into their interactions within the tumor microenvironment [45, 46]. The regulatory landscape for nanomedicine is complex, and the unique properties of nanoparticles often require extensive safety evaluations, delaying clinical applications. Regulatory bodies require comprehensive data on pharmacokinetics, biodistribution, and long-term effects before approving clinical use [7]. To overcome these challenges, several strategies have been proposed. Developing clear and harmonized regulatory guidelines can streamline the approval process, providing standardized protocols for safety and efficacy evaluations [47]. Enhanced preclinical testing using robust models that predict human responses more accurately can help identify potential risks earlier in development, thus reducing late-stage failures [48]. Additionally, adopting flexible and adaptive regulatory pathways that account for the unique characteristics of nanomedicines can facilitate their approval through case-by-case evaluations and real-world evidence [49]. International collaboration among regulatory agencies can harmonize standards and improve the efficiency of the approval process, ensuring global accessibility to safe nanomedicines [50]. Finally, engaging with the public and stakeholders, including healthcare professionals and patients, through transparent communication about the benefits and risks of nanomedicines can foster trust and promote informed decision-making. These strategies collectively aim to address the regulatory challenges of nanomedicine and expedite its clinical translation [51]. The production of AuNPs involves various synthesis methods, such as chemical reduction, seed-mediated growth, and laser ablation. These methods affect the size, shape, and surface properties of the nanoparticles. However, achieving consistent quality across batches remains a significant challenge. Variability in synthesis can lead to inconsistent therapeutic efficacy and safety, which complicates their clinical and industrial applications. Scaling up laboratory synthesis for commercial production introduces additional complexities. Traditional methods may not be easily adapted for large-scale manufacturing without compromising quality or increasing costs [52, 53]. Continuous flow reactors improve control, uniformity, and scalability, while techniques like Asymmetrical Flow Field-Flow Fractionation (AF4) help ensure batch consistency and meet quality standards [54]. One promising solution is transitioning from batch processing to continuous flow reactors. This approach offers better control over reaction parameters, ensuring uniformity in particle size and shape. Sonochemical synthesis, for example, provides high yields and can produce smaller, monodispersed nanoparticles with a narrow size distribution, which is crucial for biomedical applications. It is also easier to scale up, requires no toxic solvents, and can use biocompatible stabilizing agents [55]. Continuous flow systems offer enhanced control over reaction parameters, ensuring uniformity in particle size and shape, which is crucial for large-scale production. These systems improve scalability and reproducibility, making them ideal for consistent nanoparticle synthesis. For example, the NanoSynth Mini, an automated system using a Raspberry Pi microcontroller, has demonstrated successful production of spherical AuNPs with excellent batch-to-batch consistency through citrate-reduction techniques. Automation in the synthesis process reduces human error, and real-time monitoring systems ensure precision by adjusting deviations in reaction parameters, optimizing nanoparticle production, and maintaining high quality [56]. Achieving consistent quality in AuNP production requires careful control of reaction conditions such as pH, temperature, and reagent concentrations. Studies have shown that the concentration of trisodium citrate dihydrate and its interaction time with tetrachloroauric acid play crucial roles in nanoparticle formation. Adjusting these parameters can help standardize particle sizes, ensuring consistency across batches [57]. Another important challenge is the stability of AuNPs during storage. Factors such as aggregation or changes in surface chemistry can compromise their effectiveness as therapeutic agents. Developing stable formulations that maintain their functionality over time is critical for clinical applications [7]. The synthesis of high-quality AuNPs can be expensive due to the materials and complex processes involved. This cost may limit their widespread use in clinical practice. In addition to production costs, the overall healthcare costs associated with AuNP-based therapies can be high, including the need for specialized equipment like lasers for PTT and the monitoring of potential side effects in patients [25]. Despite the challenges in biocompatibility, targeting efficiency, regulatory approval, and production scalability, gold nanoparticles hold tremendous potential for enhancing breast cancer therapies through photothermal and photodynamic approaches. Ongoing research is crucial to optimize nanoparticle design, improve targeting efficacy, and explore scalable production methods that ensure safety and reduce costs. Addressing these obstacles will be key to translating the promise of AuNPs into effective, widely accessible treatments for breast cancer.
Conclusions and Future Perspectives
Gold nanoparticle-based phototherapy (AuNP-PT) represents a transformative approach to breast cancer treatment, leveraging the unique physicochemical properties of AuNPs for precise, minimally invasive therapies. As highlighted, AuNPs facilitate effective photothermal and photodynamic actions, overcoming limitations associated with traditional therapies like chemotherapy and radiation. The integration of AuNPs with advanced imaging, targeted drug delivery, and phototherapy modalities has demonstrated synergistic effects, particularly in addressing aggressive breast cancer subtypes like triple-negative breast cancer (TNBC). Despite these promising results, challenges such as biocompatibility, targeting specificity, regulatory compliance, and production scalability remain significant hurdles that must be systematically addressed.
Future research should prioritize the refinement of AuNP designs to enhance their biocompatibility and targeting specificity, potentially through the integration of biomimetic coatings, advanced ligands, and adaptive surface modifications. Detailed investigations into the biological pathways underlying AuNP-induced cytotoxicity will be critical for optimizing treatment protocols and minimizing adverse effects. Further advancements in imaging technologies and real-time monitoring tools will enable a more precise understanding of AuNP behavior in vivo, allowing clinicians to tailor therapies based on patient-specific tumor characteristics.
Scaling up the production of AuNPs while maintaining consistency, quality, and cost-effectiveness is paramount for clinical translation. Continuous flow reactors, sonochemical methods, and automated systems offer promising pathways to achieve these objectives. Coupled with harmonized regulatory frameworks and international collaboration, these efforts can pave the way for widespread clinical adoption of AuNP-PT. By addressing current limitations and harnessing the versatility of gold nanomaterials, this innovative approach holds the potential to revolutionize breast cancer management, offering hope for improved outcomes, reduced side effects, and enhanced quality of life for patients worldwide.
Statements and Declarations
Competing Interests
The authors declare no competing interests.
Ethics approval
Not applicable.
Data availability
Not applicable.
Funding
No external funding was received for this study.
Author Information
Sara Takallu—Department of Medical Nanotechnology, School of Advanced Medical Sciences and Technologies, Shiraz University of Medical Sciences, Shiraz, Iran;
orcid.org/0000-0001-5054-4127