Visual Abstract
Abstract
RNA-based therapeutics, encompassing microRNA (miRNA), messenger RNA (mRNA), and small interfering RNA (siRNA), offer transformative potential across diverse medical domains. miRNA, by modulating gene expression through mRNA targeting, holds promise for treating diseases like cancer and viral infections. mRNA, the carrier of genetic information for protein synthesis, has revolutionized vaccination strategies and shows promise for protein replacement therapies and regenerative medicine. siRNA, through RNA interference, provides a mechanism to silence specific genes, offering therapeutic avenues for genetic disorders, cancer, and viral infections. Despite their immense potential, challenges such as RNA instability, size, and charge hinder their clinical translation. To overcome these limitations, researchers have extensively explored the development of nanocarriers, particularly polymeric nanoparticles, for efficient RNA delivery. These platforms offer enhanced stability, targeted delivery, and controlled release, significantly improving the therapeutic efficacy and safety of RNA-based therapies. This perspective provides a comprehensive overview of polymeric nanocarriers for RNA delivery, highlighting their unique advantages, current limitations, and future research directions towards advancing the clinical translation of this promising class of therapeutics.
Overview
RNA-based therapeutics have emerged as a promising approach for treating various diseases. These therapies utilize RNA molecules, such as microRNA (miRNA), messenger RNA (mRNA), and small interfering RNA (siRNA), to modulate gene expression within cells [1–3]. RNA can be used to replace missing or dysfunctional proteins, while siRNA can be used to silence specific genes [4]. RNA-based therapies offer a flexible platform capable of inducing the expression of virtually any protein, including antigens, enzymes, and cytokines, directly within the patient’s cells [5]. This technology holds immense potential for personalized medicine, as mRNA sequences can be tailored to match the genetic profile of individual patients or rapidly modified to combat emerging infectious diseases. However, the clinical translation of RNA therapeutics faces significant challenges due to RNA’s inherent instability, large size, and negative charge. RNA is susceptible to rapid degradation by extracellular RNases, and its efficient intracellular delivery is impeded by cellular membranes that repulse large, negatively charged molecules. Effective delivery platforms are crucial to overcome these limitations and unlock the full potential of RNA-based therapies[6].
Nanostructure-based non-viral vectors have gained considerable attention in recent years as effective RNA delivery vehicles [7,8]. Compared to viral vectors, non-viral vectors are less immunogenic, have a lower risk of insertional mutagenesis, and can be produced at a larger scale with more straightforward manufacturing processes. Various cationic polymers, lipids, and cell-penetrating peptides have been developed to complex with negatively charged RNA through electrostatic interactions[9]. Advanced properties of nanoparticles, such as active targeted delivery, stimuli-responsive release, and cell-penetrating peptide-aided delivery, have made significant progress in enhancing RNA transfection efficiency focusing on developments from 2019–2024, with particular emphasis on breakthrough achievements in the last two years. For instance, cationic polymers can be engineered to condense RNA into nanoparticles that protect it from degradation while facilitating cellular uptake and endosomal escape [10].
While nanoparticle delivery methods have overcome many extracellular hurdles to RNA distribution, major intracellular obstacles following cellular uptake remain challenging to address [11]. These include efficient mRNA decondensation and endosomal escape, which are critical for ensuring that the mRNA reaches the cytoplasm intact to be translated into functional proteins. Polymeric nanostructures offer unique advantages in tackling these challenges due to their versatility, biocompatibility, and potential for functionalization. Polymers can be modified to include pH-sensitive groups that trigger the release of mRNA in response to the acidic environment of endosomes, enhancing endosomal escape and subsequent translation efficiency [12,13].
Despite the progress in developing non-viral vectors, achieving precise control over the bio-distribution of mRNA remains a critical challenge [14]. The bio-distribution of mRNA-loaded nanoparticles can significantly influence the therapeutic outcome, as off-target delivery may lead to undesired effects or reduced efficacy [15,16]. Researchers are actively exploring surface modification strategies, such as the attachment of targeting ligands, to direct nanoparticles to specific tissues or cell types. Moreover, the immunogenicity of mRNA-loaded nanoparticles is an area of ongoing investigation. Strategies to mitigate these immune responses include the use of immunomodulatory agents and the design of nanoparticles with reduced recognition by the immune system.
Parameter | Traditional Methods | New RNA Nanoparticle Methods | Improvement Factor | Ref |
Cellular Uptake Efficiency | 15–25% | 45–75% | 2–3x higher | [23] |
RNA Stability (Half-life) | 4–6 hours | >24 hours | 4–6x longer | [24] |
Target Tissue Specificity | 20–30% | 70–85% | ~3x more specific | [25] |
Transfection Efficiency | 30–40% | 60–80% | 2x higher | [26] |
Protection from RNase | Limited (<30% protected) | High (>85% protected) | ~3x better protection | [27] |
Immunogenicity | High immune response | Minimal immune response | 5x lower immune response | [28] |
Duration of Expression | 2–3 days | 7–14 days | 3–5x longer | [29] |
Payload Capacity | 2–4 kb RNA | Up to 15 kb RNA | 4x higher capacity | [30] |
Manufacturing Scalability | Limited batch size | Large-scale production possible | 10x higher throughput | [31] |
Therapeutic Window | Narrow (2–3x) | Wide (10–15x) | 5x broader range | [32] |
The choice of polymer in the design of mRNA delivery systems is also pivotal, as it influences not only the delivery efficiency but also the biodegradability and safety profile of the nano-carriers [17]. Recent research has focused on developing novel polymers with enhanced biocompatibility and degradability, which can be broken down into non-toxic byproducts after delivering their cargo. In addition to biodegradability, the mechanical properties of polymers are being tailored to improve the stability and performance of mRNA delivery systems. For instance, polymers with optimal flexibility and strength can protect mRNA from mechanical stress during circulation while allowing for efficient release upon reaching the target cells[18,19]. Another promising direction in mRNA delivery research involves the development of stimuli-responsive polymers that release their payload in response to specific environmental triggers, such as changes in pH, temperature, or redox conditions [20,21]. These smart delivery systems can enhance the precision of mRNA release, ensuring that the therapeutic mRNA is delivered at the right time and place within the body [22].
Our comprehensive review explores groundbreaking studies that demonstrate the versatility of RNA nanocarrier systems in addressing complex medical challenges across three primary therapeutic landscapes: genetic disorders, infectious diseases, and regenerative medicine.
Table 1. compares average values across multiple studies. Individual results may vary based on specific applications and conditions. Traditional methods include naked RNA delivery and basic lipofection, while new methods encompass advanced nanoparticle formulations including lipid nanoparticles, polymer-based carriers, and hybrid systems.
The field of RNA nanocarrier development is simultaneously exciting and complex, characterized by significant scientific challenges that researchers are systematically addressing. Critical obstacles such as enhancing endosomal escape mechanisms, improving RNA stability, minimizing potential toxicity, and achieving targeted cellular delivery represent key areas of intense investigation. Polymer-based systems have emerged as particularly promising platforms, with researchers exploring diverse chemical structures and innovative helper polymers that can optimize RNA complexation, protect genetic payloads, and facilitate efficient cellular uptake (Figure 1). These sophisticated nanocarrier designs aim to overcome biological barriers, ensuring that therapeutic RNA molecules can effectively reach their intended cellular targets while maintaining structural integrity and minimizing potential adverse interactions within biological systems.
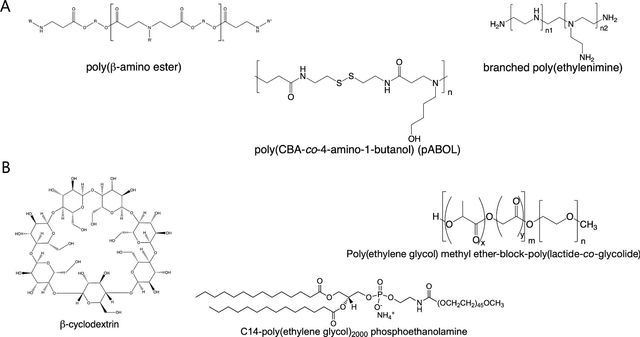
Infectious diseases
Versatile vaccination methods are crucial for combating highly adaptable viral infections. These viruses can rapidly mutate, rendering existing vaccines less effective. Therefore, it is crucial to develop vaccination strategies that can adapt to evolving viral threats [33,34]. One study addresses the critical challenge of sepsis by developing an innovative therapeutic approach using CRV peptide-modified lipid nanoparticles (CRV/LNP-RNAs) to reprogram macrophages directly in the body (Figure 2). The researchers designed a nanoparticle system that can deliver two key genetic components to macrophages: a chimeric antigen receptor (CAR) targeting MRSA and a siRNA targeting CASP11, a protein that helps bacteria evade immune responses. By enabling macrophages to specifically recognize and more effectively destroy methicillin-resistant Staphylococcus aureus (MRSA), the approach aims to overcome the limitations of current sepsis treatments. The engineered macrophages demonstrated an enhanced ability to phagocytose and internally digest MRSA, potentially offering a promising strategy for combating multidrug-resistant bacterial infections [34].
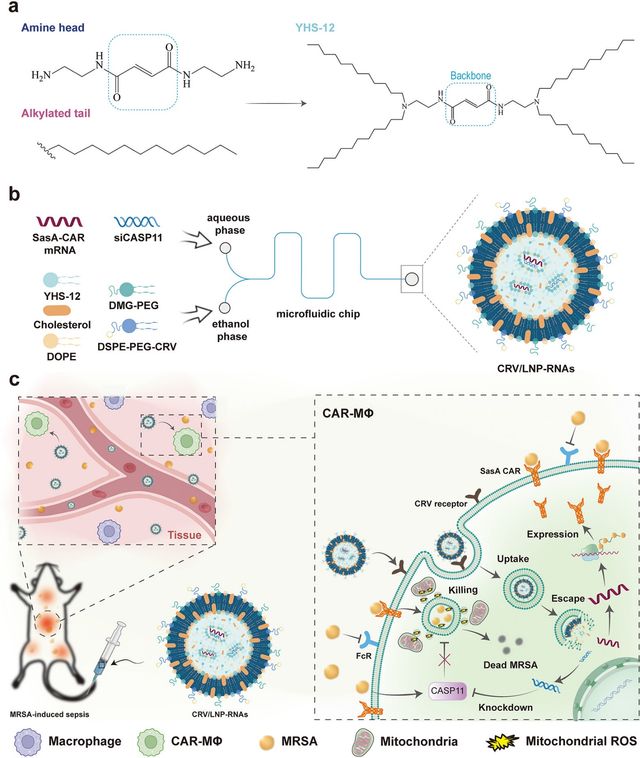
Recent advancements in nanomedicine have demonstrated a promising approach to treating idiopathic pulmonary fibrosis (IPF) through an innovative inhaled mRNA nanoformulation. The research focuses on leveraging biogenic ribosomal proteins as a sophisticated delivery mechanism for targeted therapeutic intervention[35]. As illustrated in Figure 3, the study presents a multifaceted strategy involving a novel nanocarrier system (mMMP13@RP/P-KGF) that exhibits remarkable pH-sensitive and matrix metalloproteinase–2 (MMP2)-responsive characteristics [36]. By exploiting the unique properties of ribosomal protein families and designing a precisely engineered nanoformulation, researchers have developed a potential breakthrough treatment that can potentially reverse established pulmonary fibrosis. The approach demonstrates the potential of targeted mRNA delivery to specifically modulate cellular processes, offering a nuanced and potentially transformative strategy for addressing this challenging respiratory disease.
Besides viral infection, microbial infections remain a significant challenge in biomedicine. For instance, bone infection in orthopedics, often requires prolonged antibiotic treatment and extensive tissue removal, leading to debilitating defects [37]. The emergence of antimicrobial resistance further complicates these cases. To address these issues, researchers have developed a novel, multifunctional collagen-based scaffold. This scaffold incorporates copper-doped bioactive glass (CuBG) nanoparticles, which exhibit potent antimicrobial properties without contributing to antibiotic resistance. Additionally, the scaffold delivers microRNA–138 inhibitors (antagomiRs) to stimulate osteogenesis and angiogenesis. In vitro studies demonstrated that CuBG scaffolds significantly reduced bacterial attachment, while antagomiR–138 nanoparticles promoted osteogenesis in human mesenchymal stem cells (Figure 4). In vivo, these scaffolds effectively healed large bone defects in rats and induced vasculogenesis in a chick chorioallantoic membrane model. By combining these two promising technologies, the researchers created a multifunctional scaffold capable of simultaneously combating infection and stimulating bone repair. This innovative approach offers a potential solution for treating complex bone infections and may contribute to improved clinical outcomes.
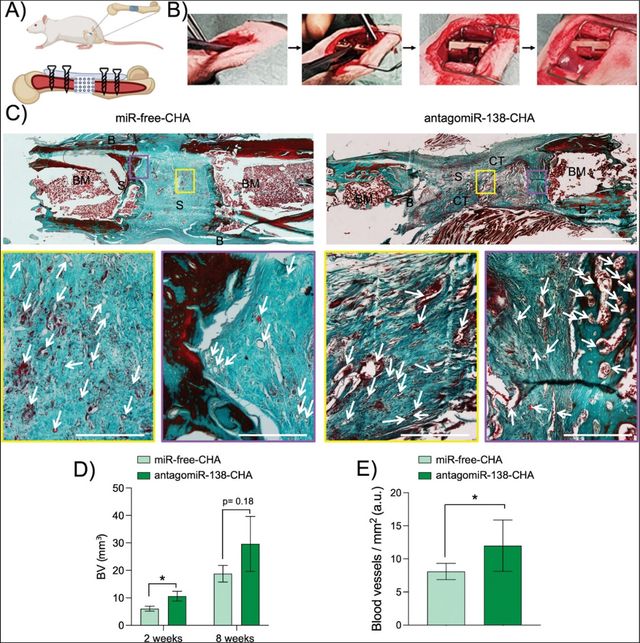
Degenerative diseases
Recent research has explored the potential of RNA-based therapies in treating degenerative diseases, with a particular focus on osteoarthritis. A notable study investigated the use of PEG-PAsp(DET) polyplex micelles to deliver mRNA encoding runt-related transcription factor (RUNX–1), a cartilage-anabolic factor crucial for regulating chondrogenesis [38,39]. Researchers investigated the therapeutic potential of 50 nm micelles delivered through intraarticular injections every 3 days for one month, starting a month after disease induction. The RUNX1-targeted treatment group exhibited promising outcomes, with inhibited osteoarthritis phenotypes and significantly enhanced RUNX1 protein expression, particularly in osteophyte-like regions. Conversely, control group samples displayed continued osteoarthritis progression, characterized by evident cartilage degradation and osteophyte formation, underscoring the potential efficacy of this targeted micelle-based intervention [40].
In a study, a new microgel gene delivery method for intervertebral disc degeneration was developed [41]. Functionalized gene nanoparticles containing siGrem1 are used in the system to improve antiapoptotic and protective properties. Targeted delivery, anti-inflammatory benefits, and reactive oxygen species (ROS) scavenging capabilities are provided by encapsulation (Figure 5. The prolonged release of siGrem1 is made possible by the microgel system, which is made up of phenylboronic acid-functionalized microspheres dynamically loaded with the HSGN. With nucleus pulposus cells, this biocompatible microgel technology efficiently reduces apoptosis, scavenges reactive oxygen species, and controls inflammation. Due to these multifunctional properties, the nucleus pulposus ECM’s metabolic balance is restored, which eventually results in delayed intervertebral disc degeneration. The research shows how well the microgel technology works in the nucleus pulposus to reduce inflammation and promote the synthesis of extracellular matrix (ECM). The nucleus pulposus’s ECM’s metabolic equilibrium may be restored by the biocompatible microgel technology. The MHSGN demonstrated notable anti-inflammatory efficacy, robust cellular affinity, and exceptional gene protection properties. The release of the HSGN from the microgel system can be triggered by the inflammatory milieu present in the degenerative intervertebral disc. These nanoparticles’ HADA can bind to NPC CD44 receptors specifically, which has anti-inflammatory properties. The Hybrid Small Guiding Nanoparticles (HSGN) are then taken up by NPCs, which makes it easier for ROS to continue to be eliminated and for siGrem1 to discharge gradually. The ability to control Nucleus Pulposus Cells (NPC) apoptosis and improve ECM production was shown by the release of siGrem1. The investigation of single-cell RNA sequencing (scRNA-seq) demonstrated that the HSGN impacted pathways linked to inflammation, synthetic processes, and cellular death. Consequently, the application of the Modified Hybrid Small Guiding Nanoparticles (MHSGN) system successfully slowed the advancement of IDD and promoted regeneration. The nucleus pulposus was recovered to reflect the features of a healthy nucleus pulposus using this microgel treatment for intervertebral disc degeneration [41].
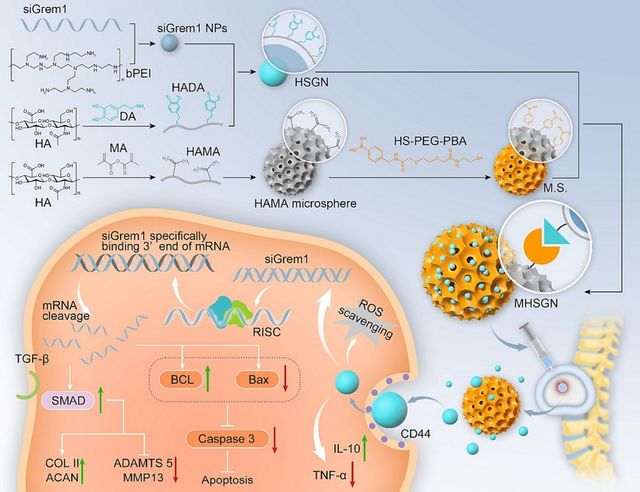
The Challenge of RNA Production Scalability
The rapid advancement of RNA-based therapies, including mRNA vaccines and RNA interference (RNAi) treatments, has revolutionized the field of medicine. However, a significant hurdle remains: the scalability of RNA production [42]. Existing methodologies for RNA production frequently entail intricate and protracted procedures, which constrain the scalability of therapies that utilize RNA [43,44]. Traditional methods of RNA synthesis, such as in vitro transcription (IVT), often require multiple steps that can be time-consuming and labor-intensive. These processes typically involve the use of plasmid DNA templates, which must be meticulously prepared and purified before transcription can even begin [45]. The purification of RNA products, which is essential to remove contaminants and ensure the integrity of the final product, adds another layer of complexity and time to the production pipeline [46]. Moreover, the scale-up of these processes poses additional challenges. As demand for RNA-based therapies increases, the need for larger quantities of high-quality RNA becomes critical [47]. However, scaling up production often leads to inconsistencies in yield and quality, as the parameters that work well at a small scale may not translate effectively to larger batches [48]. This inconsistency can result in variations in therapeutic efficacy and safety, which are unacceptable in clinical applications. Additionally, the current production methods often rely on specialized equipment and reagents that may not be readily available or cost-effective for large-scale manufacturing [49]. This limitation can hinder the ability of companies to meet the growing demand for RNA therapies, particularly in the face of public health emergencies, such as pandemics, where rapid production is essential.
To address these scalability challenges, there is a pressing need for innovative approaches and technologies that can streamline RNA production [50]. This includes the development of more efficient transcription systems, improved purification techniques, and the integration of automation and high-throughput methods. Advances in synthetic biology and biomanufacturing could also play a crucial role in enhancing the scalability of RNA production, enabling the rapid and cost-effective generation of RNA at the scale required for widespread therapeutic use [51].
In conclusion, while the potential of RNA-based therapies is immense, the scalability challenge in RNA production remains a significant barrier to their widespread adoption. Overcoming this challenge will require concerted efforts from researchers, industry leaders, and regulatory bodies to develop and implement more efficient and scalable production methodologies. Only then can we fully realize the promise of RNA therapies in transforming healthcare and addressing urgent medical needs.
Perspective
RNA is of great interest as a promising alternative for treating a wide range of regenerative and degenerative diseases [52,53]. However, several inherent challenges hinder their effective formulation and clinical application. Recent advances in the design of polymer-based delivery systems, such as the incorporation of biodegradable components and the development of novel polymer architectures, have shown promise in reducing toxicity and improving the safety profile of RNA-based therapies. Finally, the combination of RNA therapy with other therapeutic modalities, such as gene editing or protein delivery, is gaining traction as a strategy to enhance therapeutic outcomes. By co-delivering RNA with other agents, it is possible to achieve synergistic effects that can address complex diseases more effectively [54]. For example, the co-delivery of mRNA with CRISPR-Cas9 components can enable precise gene editing while simultaneously providing a therapeutic protein, offering a multifaceted approach to disease treatment [55]. These challenges include their high molecular weight, low stability, immunogenicity, short half-life, and the numerous barriers that prevent them from efficiently reaching target sites. Traditional delivery systems often fall short of fully unlocking the therapeutic potential of RNA, which has led to the development of both viral and non-viral delivery vectors.
Looking forward, the perspective in the field of RNA delivery suggests it is poised for transformative advancements. Key areas for future research include developing novel polymeric materials that enhance RNA stability and facilitate efficient cellular uptake while minimizing toxicity [56]. The integration of advanced polymeric systems with cutting-edge technologies such as nanotechnology and targeted delivery mechanisms could significantly improve the precision and efficacy of RNA therapeutics. Additionally, exploring combination therapies—where RNA delivery systems are used in conjunction with other treatment modalities like gene editing tools or small molecules—may offer synergistic benefits and address complex disease mechanisms more effectively. Interdisciplinary collaboration will be essential in overcoming current challenges and translating these innovative systems into clinical practice, potentially revolutionizing therapeutic approaches for a wide range of diseases [57].
Several polymers are under development for gene therapy, each offering unique benefits. PEG, for example, is a key component in FDA-approved drugs and is known for its ability to extend the half-life of formulations and reduce serum protein binding. Advances in combining PEG with other polymers, such as polyaspartamide, are showing promising results across various diseases [14,58,59]. Similarly, PEI continues to be a valuable polymer in creating effective polyplex micelles and complexes. Moreover, combining polymers with cyclodextrins and lipid moieties, resulting in lipopolyplexes, presents a promising area of research that warrants further investigation to fully understand its advantages and limitations.
Statements and Declarations
Authors’ Contributions
Writing Original Draft: M Moradialvand; Review & Editing: P Asadollahi
Competing Interests
The authors declare no competing interests.
Ethics Approval
Not applicable.
Data Availability
Not applicable.
Funding
No funding was received to support this work.
Authors’ Information
Madineh Moradialvand—Department of Pharmaceutical Engineering, Medicinal Plants and Drugs Research Institute, Shahid Beheshti University, Tehran, Iran;
Parisa Asadollahi—Department of Microbiology, Faculty of Medicine, Ilam University of Medical Sciences, Ilam, Iran;