Visual Abstract
Abstract
This research synthesized a three-dimensional functional polymeric network with heterocyclic bridges. Initially, cyanuric triazides and poly(acrylamide-co-acrylonitrile) were produced. A click reaction between these two components led to the formation of the three-dimensional polymeric networks (TET-PAAN), which were used as adsorbents for heavy metal ions, showing selectivity for Pb2+ ions. The polymeric networks were characterized using FT-IR, TGA, and SEM analyses. Additionally, various adsorption parameters such as pH, adsorbent dosage, adsorption time, temperature, agitation speed, and initial ion concentration were investigated and optimized. The results showed that the maximum efficient removal of the Pb2+ ions appeared at pH 7.0 (94%). The optimal adsorbent dose for the Pb2+ ions solution (50 mL) was about 5 mg/50 mL (70% to 96%), and the equilibrium time for the synthesized nanocomposite was found to be about 15 min.
Introduction
With the speedy improvement of the production industry, severe environmental issues are emerging. Among those issues, pollutants from heavy metals, inclusive of Pb, Cd, Cr, and as, offer predominant worries for human health. Excess heavy metals in water and soil can input the human frame via meals and water intake and may accumulate, inflicting dangerous effects on the kidneys, liver, and anxious system. Many procedures for the elimination of Pb2+ from business wastewater have been reported, amongst which adsorption is taken into consideration as a green method due to the fact it’s far more cost-effective, calls for moderate working situations, and has an excessive efficiency [1,2]. The aromatic six-membered ring of s-triazines with an alternating order of C- and N-atoms is present in many compounds. Some, like melamine, cyanuric chloride, cyanuric acid, and various derivatives, are produced industrially on a large scale [3-5]. Functionalizing an organic nitrile followed by cyclization (2+2+2-cycloaddition) of three CN groups is a significant option; another is the substitution of the halogen atoms of cyanuric halides and related derivatives. 1,3,5-Triazine derivatives have various applications in pharmaceuticals, textiles, plastics, and rubber industries, functioning as pesticides, dyestuffs, optical bleaches, explosives, and surface-active agents. The chemistry of these compounds has been extensively researched [6-11].
Improvement of important techniques for preparing various substances remains a significant challenge in modern synthetic organic chemistry, focusing on selectivity, mildness, enhanced efficiency, and reduction of toxic reagents and by-products. Consequently, there has been a notable emphasis on exploring novel 1, 3, and 5-triazine derivatives as reagents in organic synthesis. In recent years, the removal of heavy metal ions from both natural waters and wastewater has become increasingly crucial in addressing and mitigating industrial and environmental waste issues [12-15]. Among the different toxic metal ions, lead stands out as a major pollutant harmful to aquatic life and detrimental to human health. It can lead to serious conditions like hepatitis, anemia, nephritic syndrome, mental retardation, headaches, dizziness, irritability, and muscle degradation. Therefore, it is crucial to eliminate this ion or reduce its concentration to a safe level [16-19]. Various technologies, including chemical precipitation [20], a ferrite method [21], a chelation–precipitation method [22,23], an ion exchange [24], adsorption [25], membrane separation [26], an electrochemical method [27], and biological flocculation [28], have been used for the removal of highly toxic heavy metal ions from water. Among the various solid adsorbents, polymeric chelating resins are widely used in the removal of metal ions due to their high adsorption capacities and selectivity [29- 33]. Several criteria such as specific and fast complexation of the metal ions as well as the reusability of the adsorbent are important in the design of metal-chelating polymers.
The selectivity and separation of elements from aqueous solutions depend both on elemental speciation and on the chelating properties of the polymer. For example, polystyrene-bound hexaketone [34] and polyallyamine phosphonic acid [35] are highly selective for extracting UO22+ from seawater. Polystyrene-based chelating resins with oxime and diethylamino functional groups [36] have a higher selectivity for Cu2+ and UO22+. Polyacrylamide (PAAM) is a well-known water-soluble polymer containing large numbers of amide groups [37,38], and it has been grafted on various adsorbent materials. [39,40] Polyacrylonitrile (PAN) has been recognized as a highly efficient material for the removal and enrichment of heavy metals [41]. In recent years, Baghayeri et al. have carried out studies on the subject of the identity and absorption of heavy metals in aquatic environments. such as: synthesizing an electrochemical sensing protocol primarily based totally on the amendment of a GCE with GO-Fe3O4-PAMAM nanocomposite for simultaneous detection of Pb(II) and Cd(II) [42], introducing the synthesis and electrochemical utility of glutathione functionalized magnetic nanocomposite (GSH@Fe3O4) for the improvement of a simple, stable, and selective sensor for heavy metallic ion detection in real-existence samples [43], and synthesize polyamidoamine dendrimer functionalized magnetic nanoparticles (Fe3O4@G2-PAD) for the measuring of Pb2+ and Cd2+ ions in environmental waters [44].
In this study, the goal of work is the synthesis of a polymeric network with heterocyclic bridged as a novel product with the ability to absorb various compounds such as pb2+. For this purpose, we used click reaction for the creation of heterocyclic compounds in the network formation process. So, cyanuric chloride is reacted with NaN3 to synthesize cyanuric triazides. Subsequently, the poly (acrylamide-co-acrylonitrile) is produced through free radical polymerization. In the final step, a click reaction is conducted between the poly (acrylamide-co-acrylonitrile) and cyanuric triazides to synthesize three-dimensional polymeric networks (TET-PAAN), with heterocyclic bridges. This product exhibits high specific surface area, making it a superior adsorbent with higher adsorption rates and capacities compared to materials such as resins, foams, and conventional fibers. The TET-PAAN is utilized as a selective adsorbent for Pb2+ ions, and various analyses, including FT-IR (Fourier transform infrared spectroscopy), TGA (Thermogravimetric analysis), and SEM (Scanning electron microscopy) are performed to characterize the prepared polymeric network.
Experimental
Materials
Cyanuric chloride was purchased from Alfa-Aesar, and acrylamide and acrylonitrile (≥99% Merck) were used without further purification. NaN3 was purchased from Merck. \(\alpha\), \(\alpha’\)- azobis (isobutyronitrile) (AIBN) (≥98% Merck) was recrystallized from hot (50 °C) methanol and kept away from light before use. The analytical-reagent grade Pb(NO3)2 and other organic solvents and inorganic chemicals were purchased from Merck.
Characterization methods
The FT-IR spectra were captured using a Thermo Nicolet NEXUS 670 Fourier transform infrared spectrophotometer. SEM images were acquired from a LEO 1430 VP (Leo Electron Microscopy Ltd, UK). Samples underwent thermogravimetric analysis (TGA) using the METTLER 851 (Switzerland), scanning up to 600 ℃ at a heating rate of 10 °C min–1 under N2 atmosphere. Metal ion concentrations in the solution were determined with an atomic absorption spectrophotometer (AAS) (Analytic Jenanov AA 400).
Synthesis of heterocyclic cyanuric triazide
Cyanuric triazide is synthesized by reacting sodium azide and cyanuric chloride in an aqueous acetone solution [45]. To achieve this, in a 100 mL beaker with a magnetic stirrer bar, NaN3 (2.5 g) was dissolved in H2O (10 mL), followed by the addition of acetone (15 mL). The solution was heated to 50 °C, then C3N3Cl3 (1.55 g) was introduced, and the reaction was allowed to proceed with stirring for two hours. The acetone layer was evaporated under a hood at 50 °C, resulting in the gradual formation of a white precipitate. To purify the product, the white precipitate was transferred to ice water (150 mL) with vigorous magnetic stirring. After decanting the solution and filtering the precipitate, it was dried in a vacuum oven.
Synthesis of poly (acrylamide-co-acrylonitrile)
In a 100 mL two-necked round-bottom flask equipped with a stirrer and inlet and outlet of inert gas, DMF (50 mL), acrylamide (1.47 g, 0.0188 mol), and acrylonitrile (2 g, 0.0376 mol) were introduced. The flask contents were degassed for 30 minutes, following which AIBN (0.019g, 0.0564 mol) was included. The reaction mix was stirred under an argon atmosphere at 80 °C for 24 hours. Subsequently, after the specified duration, the flask contents were mixed with 50 mL H2O as a nonsolvent to yield the final product as a precipitate. The precipitate was filtered, washed multiple times with DMF, and THF, and dried in a vacuum oven overnight.
Synthesis of the TET-PAAN network
In a 50 mL round-bottom flask, combine DMSO (50 mL), poly(acrylamide-co-acrylonitrile) (0.4327 g), and cyanuric triazide (0.2041g, Mw 204.12). Then add CuSO4 (0.043276 g) as a catalyst. Stir the reaction mixture and reflux at 110 °C for 120 hours. After this period, transfer the flask contents to a 100 mL saltwater solution to yield the final product as a precipitate. Filter the precipitate, wash it multiple times with DMSO and DMF, then dry it in a vacuum oven overnight.
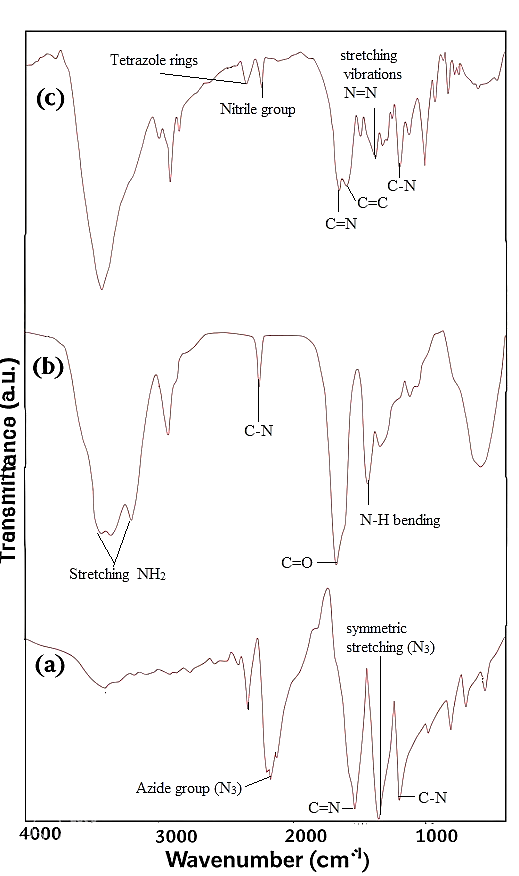
Results and Discussion
Synthesis of heterocyclic cyanuric triazide
Metallic or covalent nitrides are typically challenging to produce due to the utilization of stable molecules like ammonia or N2 as nitrogen sources. Azides (-N3) are a known class of compounds that serve as accessible nitrogen sources, with NaN3 being particularly useful in various metal nitride syntheses. Although azides are commonly unstable, the 2,4,6-triazido–1,3,5-triazine, also known as cyanuric triazide, stands out as a moderately stable molecular azide. Initially synthesized and structurally characterized in the early 21st century, its structure has been recently reexamined. The synthesis involved the reaction of cyanuric chloride with three equivalents of sodium azide in acetonitrile solution, as illustrated in Scheme 1. The FT-IR spectra of cyanuric triazide are depicted in Figure 1a. Within this spectrum, the peak at 2144 cm−1 corresponds to the asymmetric stretching vibration of the azide group (N3), while a strong peak at 1347 cm−1 represents the symmetric stretching vibration of this group. Additionally, the prominent absorption peaks at 1188 cm−1 relate to the stretching vibration mode of C–N, and the peak at 1529 cm−1 corresponds to the conjugated C = N and C = C species.
Synthesis of copolymer
For synthesizing the copolymer, the radical copolymerization of acrylamide and acrylonitrile in the presence of the AIBN as the initiator in the DMF solution was carried out. The FT-IR spectrum of the copolymer is presented in Figure –1b. As can be seen in this figure the peak at 2244 cm–1 was related to the nitrile group of the copolymer, the peak at 1671 cm–1 belonged to the carbonyl group, and two peaks at 3431 cm–1 and 3353 cm–1 are attributed to stretching of NH2 groups in the copolymer structure.
Synthesis of the TET-PAAN network
The TET-PAAN network was obtained from the click reaction of a poly (acrylamide-co-acrylonitrile) with cyanuric triazide and pendant CN groups of the copolymer. In this reaction, cyclization (2+2+2-cycloaddition) of three N3 groups of cyanuric triazide, and CN groups of the copolymer were carried out. The accomplishment of this reaction, in addition to the three-dimensional polymeric network, that appeared, also caused tetrazole rings to be formed, which can increase the ability of the TET-PAAN network for the adsorption of heavy metal ions. Scheme 2 shows the formed tetrazole ring as the result of the click reaction in the produced polymeric network, and the FT-IR spectrum of TET-PAAN is presented in Figure 1c.
In this figure, the peak at 2244 cm–1 corresponding to the nitrile group of the poly(acrylamide-co-acrylonitrile) copolymer disappeared, while peaks associated with tetrazole rings emerged between 2634 cm–1 and 2854 cm–1. Additionally, the peak at 1604 cm–1 is indicative of the stretching vibration of the C = N group in the tetrazole ring, and peaks at 1509 cm–1 and 1397 cm–1 are attributed to the stretching vibrations of the N = N and C-N groups within the tetrazole rings of the polymeric network structure.
Structural characterization
To examine the thermal stability of the prepared polymeric network, TGA analysis was conducted (Figure 2). The graph illustrates a sequential series of events. Initially, moisture loss occurs, continuing up to approximately 150 ℃. Subsequently, a second phase commences around 180 ℃ and is associated with the separation of cross-linking and pendant groups. The third stage, spanning 250 ℃ to 450 ℃, corresponds to the decomposition of cyanuric triazide and the combustion of the aromatic six-membered ring. Above 450 ℃, the primary polymer structure is degraded, extending up to around 700 ℃.
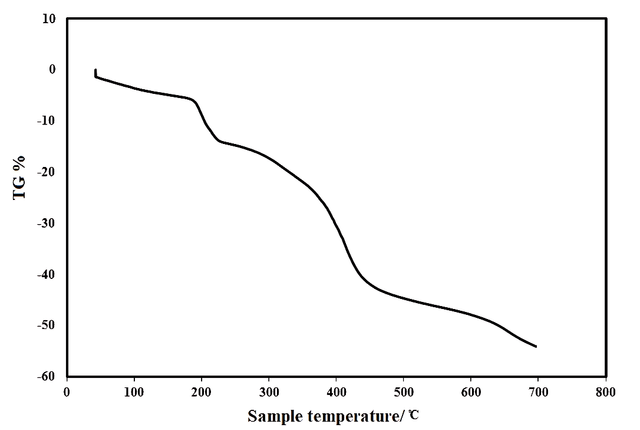
Figure 3 shows the SEM images of the sample. The SEM image of the TET-PAAN illustrated a particle-like architecture. It was evident that the surface of the material was coarse because it was covered with interlaced cyanuric triazide flakes.
Adsorption study of the click reaction adsorbent
The Optimization of Parameters
Effect of pH value
The pH value of the media is one of the most important factors in the adsorption of metal ions. It is well known that Pb2+ions are present in several forms in media depending on the pH solution: these are Pb2+, Pb(OH)+, Pb(OH)2, and Pb(OH)3. The effect of pH on the adsorption of Pb2+ ions with the TET-PAAN network was investigated in pH ranges between 2.0 and 7.0 at 25°C with an initial Pb2+ ions concentration of 10 mg L–1. In the basic pH, the lead ions were in the insoluble form therefore, we didn’t work in the basic area. The results showed that the adsorption of Pb2+ ions in solution completely depends on pH, in fact at an acidic pH the H+ ions compete with the Pb2+ ions to reach functional groups of the synthesized composite. Thus, the adsorption of Pb2+ ions in acidic media is low. The maximum efficient removal of the Pb2+ ions appeared at pH 7.0 (94%). Therefore, pH–7 appeared to be the optimal condition and was chosen as the optimal pH for the following experiments. The effect of pH on lead adsorption is shown in Figure 4a.
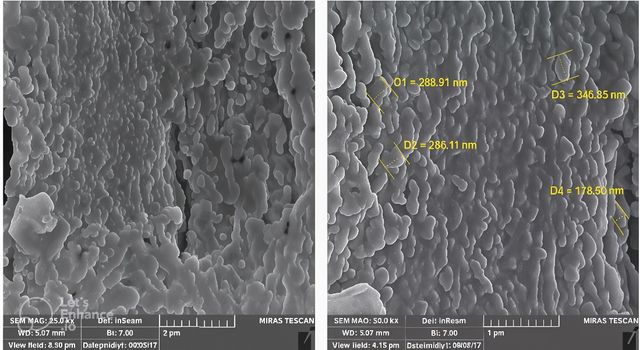
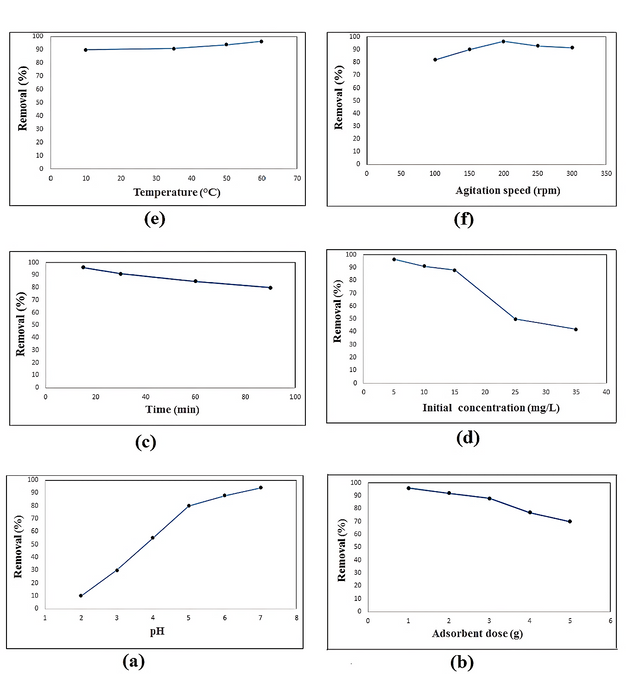
Effect of adsorbent dosage
To determine the optimal adsorbent dose for the Pb2+ ions solution (50 mL) at an initial concentration of 10 mg L–1, various adsorbent dosages (0.005, 0.01, 0.02, 0.03, 0.04, and 0.05 g) of the synthesized TET-PAAN network were added. The results of the experiments are presented in Figure 4b. It can be seen that the efficient removal of metal ions was about 5 mg/50 mL. (70% to 96%)
Effect of adsorption time
The contact time between the metal ions and adsorbent plays an important role in the adsorption process and reveals the minimum time required for the removal of the maximum percentage of pollutants from the media. Figure- 4c shows the contact time vs. the removal percentage curves. It can be observed that the uptake of Pb2+ ions was rapid, and a large amount of this toxic ion was removed within a few minutes (15 min). After this time there was no significant change in terms of the amount of adsorption due to the saturation of the active sites on the absorbent surface. Therefore, the equilibrium time for the synthesized nanocomposite was found to be about 15 min.
Effect of initial concentration
The Pb2+ ions solutions with different initial concentrations (5, 10, 15, 25, 35, and 50 mg L–1) were used to study the effect of concentration variation on the removal of metal ions by a 0.005 g L–1 adsorbent at pH 7 and temperature 298 K. It can be seen from Figure- 4d that the removal yield decreased from 96.5 to 42% as the initial Pb2+ ion concentration increased. Therefore, at a higher initial concentration of Pb2+ ions, the adsorption was decreased due to the active sites of the adsorbent being rapidly occupied by metal ions, and the number of accessible adsorption sites was reduced [46].
Effect of solution temperature
Temperature plays an important role in the adsorption process as it provides valuable information on three basic thermodynamic parameters; the standard Gibbs free energy, enthalpy, and entropy changes. The changes in these parameters were used to characterize the adsorption thermodynamics. In this study, the Pb2+ ions adsorption experiment was performed with varying temperatures from 10 to 60 using 0.005 g of adsorbent at pH 7. The removal percentage of Pb2+ ions that were adsorbed onto the TET-PAAN network at different temperatures is shown in Figure- 4e.
The results showed that adsorption increased slightly from 90 to 96.5% when the temperature increased from 10 to 60. This result showed that the adsorption of the lead ions was an endothermic process. The increase in the adsorption with the increase in temperature may be due to the increase in the mobility of the ions, which can probably increase the number of ions for interaction with active sites [47].
Effect of agitation rate
Agitation speed is a key factor influencing adsorption performance and solute distribution in a solution. To investigate the impact of agitation rate on the efficiency of Pb2+ ion removal, the agitation speed was varied from 100 to 300 rpm while keeping other conditions constant. Figure - 4f illustrates that increasing the agitation rate to 200 rpm led to a higher adsorption capacity. This is attributed to heightened turbulence, which facilitated greater diffusion of the adsorbate ions into the internal surface of the adsorbent, consequently reducing the boundary layer thickness around the adsorbent particles. [48].
Further increase in the agitation rate to 250 and 300 rpm reduced the adsorption capacity because the extreme turbulence caused a decrease in the interplay time between the adsorbent and adsorbate ions. An agitation speed of 200 rpm was therefore selected as optimal.
Table 1 displays a comparison table of the maximum adsorption capacity (Qm) for Pb2+ ion removal between the findings of this investigation and those reported in the literature.
Adsorbent | Experimental Conditions | Removal (%) | Reference |
(TET-PAAN) | pH 7.0, 15 min., 5 mg/50mL initial Pb2+ | 96% | This Research |
P-type zeolite | pH 5.0, 2 min., 7.5mg/L initial Pb2+ | 97% | [49] |
Di-acrylate Pluronic P123 hydrogels | pH 7.0, 12h., 7.5mg/L initial Pb2+ | 85.3% | [50] |
5-sulfosalicylic acid-modified lignin | pH 5.85, 12h., 5g/L initial Pb2+ | 99.8% | [51] |
Conclusion
A three-dimensional functional polymeric network was synthesized in this study. The resulting product was used as an effective adsorbent for heavy metal ions, particularly Pb2+. Various analyses, including FT-IR, TGA, and SEM, were performed to characterize the prepared polymeric network. Additionally, the impact of different adsorption parameters, such as pH, adsorbent dosage, adsorption time, temperature, agitation speed, and initial ion concentration, was investigated and optimized. The results showed that the polymeric network exhibited exceptional efficiency in Pb2+ ion removal from aqueous solutions. The maximum efficient removal of the Pb2+ ions appeared at pH 7.0 (94%). The optimal adsorbent dose for the Pb2+ ions solution (50 mL) was about 5 mg/50 mL (70% to 96%), and the equilibrium time for the synthesized nanocomposite was found to be about 15 min. Also, the results indicated that adsorption increased from 90% to 96.5% as the temperature rose from 10℃ to 60℃, confirming that lead ion adsorption is an endothermic process. This increase may result from enhanced ion mobility, which elevates the number of ions interacting with active sites. However, the removal yield fell from 96.5% to 42% with a rising initial Pb2+ ion concentration. At higher initial concentrations, adsorption decreased due to the rapid occupation of the adsorbent’s active sites by metal ions, reducing the number of available sites for further adsorption. The superior performance of the synthesized polymeric network in heavy metal removal opens avenues for its application in various industrial and environmental contexts. These findings significantly advance the field of advanced materials for environmental applications, demonstrating the potential of engineered polymers in addressing water pollution challenges. This work advances the field of polymeric network-based environmental remediation technologies, providing a promising solution for sustainable water treatment practices.
Acknowledgments
The authors wish to acknowledge financial and spiritual support from Urmia University.
Statements and Declarations
Authors' contributions
Mahsa Ensafi Aval is a Ph.D. student who did the experimental work; Sedigheh Ehsanimehr wrote the original draft of the manuscript; Peyman Najafi Moghadam was a supervisor and revised the manuscript; and Mohamad Mehdi Baradarani was a supervisor and revised the manuscript.
Competing interests
The authors declare no competing interests.
Ethics approval
Not applicable
Data availability
Not applicable. All the data in the manuscript file is available.
Funding
This research received no specific grant from funding agencies in the public, commercial, or not-for-profit sectors.
Authors’ Information
Mahsa Ensafi Avala—Department of Organic Chemistry, Faculty of Chemistry, Urmia University, Urmia, Iran;
Sedigheh Ehsanimehr—Department of Organic Chemistry, Faculty of Chemistry, Urmia University, Urmia, Iran;
Peyman Najafi Moghadam—Department of Organic Chemistry, Faculty of Chemistry, Urmia University, Urmia, Iran;
Mohammad Mehdi Baradarani—Department of Organic Chemistry, Faculty of Chemistry, Urmia University, Urmia, Iran;